
- science goal
The dual-frequency SAR will enable a host of applica-
tions like locating and quantifying water–ice in the lunar
polar regions; understanding the scattering characteristics
of the lunar surface and subsurface features; mapping the
lunar craters and other geological features, especially in
the polar regions, with finer details; and retrieving lunar sur-
face parameters like dielectric constant and surface rough-
ness. These are elaborated in the following sub-section
Detection and estimation of water–ice
Previous ground-based and spaceborne observations sug-
gest permanently shadowed polar regions as potential zones
for presence of water–ice on the Moon. Circular Polariza-
tion Ratio (CPR), an important parameter derived from
the radar measurements, has been traditionally used to
detect the presence of water–ice. MiniSAR and MiniRF
instruments have operated in hybrid-polarimetric mode
from which Stokes’ vectors and its daughter products eg.,
‘degree of linear/circular polarization’ and CPR were
derived.
During analysis of MiniSAR data, high CPR values
were observed both inside and outside of lunar craters in
polar as well as equatorial regions. The same was also
observed in permanently shadowed as well as sunlit areas.
The incidence of high CPR can be either due to double-
bounce scattering induced by surface roughness or due to
dielectric inhomogeneity caused by ice particles in lunar
regolith, as explained by the Coherent Backscatter Opposi-
tion Effect (CBOE) (Spudis, 2006). This analysis remained
inconclusive due to limited characterization of target scat-
tering mechanism from hybrid polarimetric data. A pro-
posed solution to this problem uses the Vector Radiative
Transfer (VRT) model that explains the complete scatter-
ing behavior of the target based on its physical property
and composition. The VRT model (Fa et al., 2011) requires
full-polarimetric data (rather than hybrid-polarimetric
data) as input. Full-polarimetric data contains more com-
prehensive scattering information for any arbitrary polar-
ization state (e.g., circular, linear and hybrid modes).
This enables certain target decompositions that are model
based (Freeman and Durden, 1998) and Eigen–vector
based (Cloude, 1983; Cloude and Pottier, 1997), yielding
more information about a target than m–d or m–v decom-
position (Raney, 2007a, 2012). The final result is a much
less ambiguous detection of water–ice; thus full-
polarimetric modes of imaging have been planned in this
mission in addition to those of hybrid polarimetry.
Surface roughness derived from SAR measurements is a
function of incidence angle (Fa et al., 2011). Observations
at multiple incidence angles planned in this mission will
help further in resolving high CPR ambiguities arising
due to roughness scattering and volume scattering. Imag-
ing with multiple viewing angles will also help in estimating
the volume of ice and the depth at which it is present.
The major differences between L-band and S-band fre-
quencies are in their ‘penetration depths’ and ‘roughness
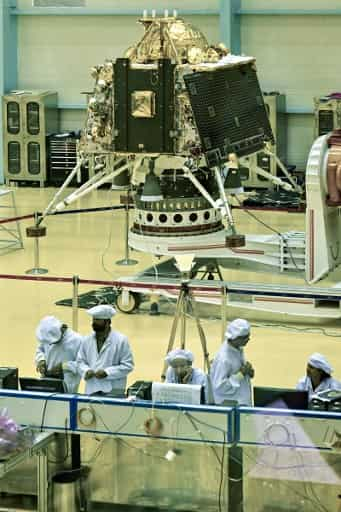
sensitivities’. shows the simulated penetration depths
(using Integral Equation Models, IEM) in lunar surface
corresponding to L- and S-band signals as a function of
FeO + TiO2 content with different bulk densities. The pen-
etration depth at L-band is almost twice of that at S-band.
Incidentally, the penetration depths of L- and S-bands at
low FeO + TiO2 content of regolith correspond to the
regolith thickness of lunar Mare and Highlands (Terrae)
region. Hence these two frequencies can be used together
to estimate the depth of sub-surface ice particles and to
derive vertical profile of regolith in the crater floor.
Due to the differences in dielectric constant (e) values of
ice, lunar regolith and lunar rocks, the backscattering coef-
ficients (r) at L- and S-band frequencies corresponding to
these materials are different. A multi-layer scattering model
(using IEM) has been developed to estimate the backscat-
tering coefficients corresponding to various compositions
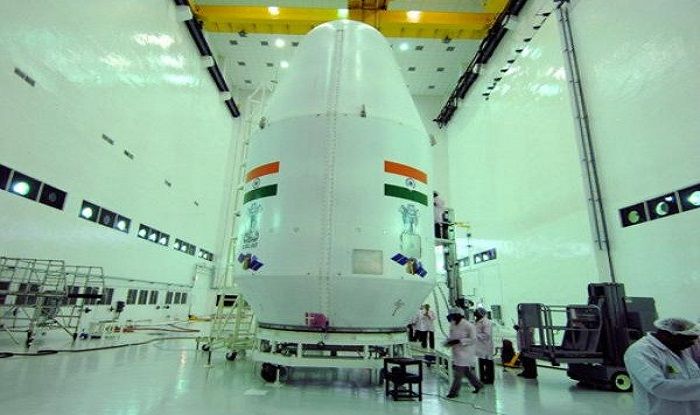
2.2. Dielectric constant and surface roughness estimation
Dielectric constant estimation is vital for understanding
the scattering contributions from the material distribution
over lunar surface that gives valuable information about
the origin and evolution of lunar features. The dielectric
constant estimation will also help in detection of minerals
that contain titanium, iron and water in the surface layers
of the moon. Various empirical and semi-empirical models
are used for dielectric constant estimation of planetary
bodies. Campbell et al. (2002) suggested a dielectric inver-
sion model for rock-poor mantling dust based on the nor-
malized ratios between the horizontal and vertical

where u is the incidence angle.
Considering some of the smooth crater fills in the lunar
equatorial region as rock-poor mantling dust surface, the
inversion model was applied on simulated linear polariza-
tion data from Chandrayaan-1 MiniSAR and encouraging
results were obtained. The study showed that dielectric
constant is a valuable parameter to identify material depos-
its on the crater floors which might indicate the origins of
the craters. For example, craters originating from meteoritic
impact and volcanic activities showed different dielectric
Simulated results showing SAR backscatter in HH-polarization in
L- and S-bands over ice in regolith and buried rocks as a function of
incident angle in lunar surface conditions.
Variation of lunar polar ice detection (LPID) index for regolith
mixed with rock particles and regolith mixed with ice particles on a
bedrock base, with respect to incidence angle; simulation considers HH-
polarization. The input parameters and their values used in the IEM:
Penetration depth = 5 m, Volume fraction of discrete scatterers = 0.01,
Radii of scatterers = 2 cm, Dielectric constants of: Ice = 3.15 j * 0.00;
Regolith layer = 2.7 j * 0.003; Buried Rocks = 6.0 j * 0.01; Bed
Rock = 6.0 j * 0.05.
constant distributions within their floors. However, the
Chandrayaan-1 MiniSAR could only map a few sites in
the lunar non-polar regions where measured dielectric con-
stant values were available for validation. As
Chandrayaan-2 dual-frequency SAR is expected to cover
polar as well as non-polar regions in full polarimetric
mode, it will facilitate development of dielectric constant
models that can be validated with observations from
Apollo missions.
Chandrayaan-2 dual-frequency SAR data with steep
(10) and moderate (35) incident angles can also be uti-
lized to estimate surface roughness of lunar regolith.
Dependence of back-scattering coefficient on surface
roughness is well known, and its sensitivity for L-band
and S-band are different as shown in Fig. 4. Hence, these
two frequencies can be used together at low and moderate
incident angles to derive roughness in the lunar surface.
2.3. Investigation of geo-morphological features
The lunar surface is marked by signatures of geo-
morphological evolution through time by activities like
meteoritic impact, volcanic eruptions and solar winds.
Such features carry significant amounts of information
about the origin and evolution of lunar crust and any phys-
ical processes on the lunar surface. While many of these
geo-morphological features have been studied and associ-
ated processes understood through optical sensors by pre-
vious lunar missions, an orbiting SAR is expected to
provide a rich augmentation of information owing to its
different target interaction mechanisms and surface pene-
tration capability. Using Chandrayaan-1 MiniSAR data
many geo-morphological features pertaining to lunar Polar
Regions were studied and mapped. However due to the
relatively poorer resolution of MiniSAR data, finer details
of the lunar morphological features could not be studied.
With high resolution modes of Chandrayaan-2
dual-frequency SAR, such features can be studied with
finer details of their structures and compositions, and
high-resolution lunar morphological maps can be
generated.
The high resolution data will also facilitate crater age
determination based on size-frequency distribution of smal-
ler craters and secondary craters on the floors of larger
craters.
Further, the advantages of high-resolution, steep incident
angles and enhanced radiometry planned in dual-frequency
SAR, will facilitate high resolution crater floor mapping.
This will play an important role in characterizing scattering
mechanisms in the areas where water–ice may exist.
2.4. Quantitative estimation of regolith thickness and
distribution
The emissivity corresponding to lunar surface materials
(in microwave region, as measured by radar brightness
temperature) can provide valuable information about the
composition of the regolith over lunar surface. The bright-
ness temperature as measured at higher wavelengths such
as S- and L-band correspond to emitted signals from a
thick layer depending on the penetration depth at those
wavelengths and is likely to provide information about
the regolith depth, distribution and its characteristics.
Change ́ 1 provided the first global map of regolith thick-
ness using multichannel microwave radiometer (Fa and
Jin, 2010). Chandrayaan-2 dual-frequency SAR can be
operated in passive mode over selected regions in lunar
polar and non-polar regions and will provide possible
opportunity for quantitative estimation of regolith distri-
bution and the associated ilmenite (FeO + TiO2) and 3
He
deposits. In this context, empirical relationships are being
developed for inversion of brightness temperature to rego-
lith thickness through three layer scattering model (Fa and
Jin, 2007).
Based on the above considerations, the science objec-
tives of Chandrayaan-2 dual-frequency SAR may be sum-
marized as:
(a) Detection and estimation of water–ice deposits in the
permanently shadowed regions of the moon, using
dual-frequency and full-polarimetric imaging at mul-
tiple viewing angles.
(b) Regolith dielectric constant and surface roughness
estimation over lunar surface using multi-frequency
data.
(c) Investigation of geo-morphological features espe-
cially in the polar regions and preparation of geo-
morphological maps and high resolution crater floor
maps.
(d) Quantitative estimation of regolith thickness and dis-
tribution over selected regions using data from dual-
frequency radiometer mode.
. Simulated roughness sensitivity of L-band (1.25 GHz) and S-band
(2.5 GHz) signals as derived using IEM for typical lunar surface
parameters. (e =5+ j (0.01); incident angle = 25).
630 D. Putrevu et al. / Advances in Space Research 57 (2016) 627–646
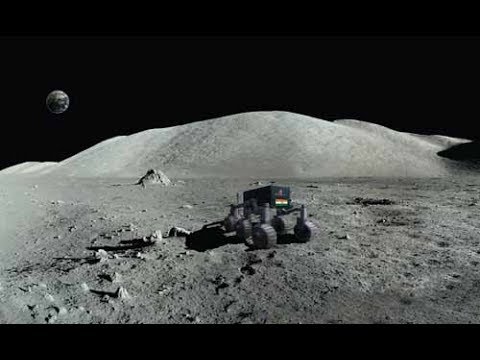
- Instrument features
The objectives outlined above need the dual-frequency
SAR to be configured with the following features.
3.1. Wide span of incidence angles
The system is designed for a wide range of angles of inci-
dence, from 9.6 to 36.9. This will enable the SAR to col-
lect information on the scattering properties of
permanently shadowed regions, especially near the lunar
poles, at multiple viewing angles for a rich characterization
of surface properties. Also the steep to moderate incidence
angle variability is expected to help in resolving ambiguities
related to the interpretation of certain incidences of high
CPR in Chandrayaan-1 MiniSAR data (Spudis et al.,
2010a), as discussed in the previous section. The wide span
of incidence angles allows for detailed analysis of target
scattering as explained below:
Previous studies using the Earth-based Arecibo radar
data indicated that the most important factor that influ-
ences the radar backscattering coefficients and CPR is the
radar incidence angle (Campbell et al., 2010; Campbell,
2012). The received radar signal is a sum of polarized
and depolarized radar backscattering coefficients. The
polarized radar echo is dominated by surface scattering
at small incidence angles (<20), while it is dominated by
scattering from buried rocks (i.e., volume scattering) at lar-
ger incidence angles. The depolarized radar echo is domi-
nated by scattering from buried rocks at all incidence
angles. CPRs corresponding to surface and subsurface
scattering are negligible at incidence angles near 0 but they
increase somewhat with increasing incidence angle, and
then decrease with incidence angles beyond 50 (Fa et al.,
2011). In this way, analyzing the received radar signal at
shallow and higher incidence angles helps in identifying
the dominant scattering mechanism (e.g., surface vs volume
scattering) at a given region.
Since large-scale surface slopes change the local inci-
dence angle and polarization state of the incident radar
wave, they also modulate the received radar backscatter
and CPR values. For example, in the case of a bowl-
shaped crater, shadowing effects should occur only for
the incidence angles greater than 65 (Fa et al., 2009). Since
most surface slopes are less than 30, the incidence angles
associated with the Chandrayaan-2 SAR (9.6–36.9) are
not large enough to cause shadowing effects for typical
lunar features.
3.2. Hybrid and full polarimetry capability
The dual-frequency SAR instrument is configured to
operate in either hybrid (circularly polarized transmission
and linearly polarized reception, (Raney, 2007b)) or full
polarimetric mode. In hybrid polarimetric mode, horizon-
tal (H) and vertical (V) polarized signals are transmitted
simultaneously with a relative phase offset of 90, which
effectively results in circularly polarized transmission. The
reception of the backscattered signal is, however, linear
H & V components. In pulsed radar systems, the hybrid-
polarimetric mode allows harnessing some of the advan-
tages of polarimetry without necessitating a high
Pulse-Repetition Frequency (PRF). A high PRF pushes
data rate up and compromises on either swath or range-
coverage. The hybrid polarimetry mode generated remark-
able results in earlier missions like the Radar Imaging
Satellite (RISAT-1) of ISRO for Earth observation (sample
images in Fig. 5) and the MiniSAR of Chandrayaan-1 for
lunar imaging. Processing hybrid polarimetry data necessi-
tates a certain adaptation of decomposition methods, like
m–d or m–v decomposition (Raney, 2007a; Raney et al.,
2012), to extract polarimetric content (using four-element
Stokes’ vector) of the scene.
Full-polarimetry, on the other hand, resorts to inter-
leaved horizontal (H) & vertical (V) transmission each at
the designated PRF (corresponding to Doppler bandwidth)
and reception of all four polarization combinations – HH,
HV, VV and VH, thereby doubling the data rate vis-a`-vis
that of hybrid polarimetric mode. However, full-
polarimetric mode, regarded to be the gold standard
among polarimetric radars, allows extraction of the com-
plete 4 4 Stokes’ matrix, considered to be important for
certain observations, and therefore, configured for the
dual-frequency SAR.
The hybrid polarimetry concept (originally from Min-
iSAR and MiniRF designs) was derived from conventional
Earth-based radars which measure CPR by transmitting
right- or left-circular polarization and receiving both senses
of circular polarization, resulting in same-sense circular
(SC) and opposite-sense circular (OC) image pairs. Even
though the main objective of MiniSAR and MiniRF radars
was to resolve the longstanding issue of detecting water–ice
in the permanently shadowed regions near the lunar poles,
the hybrid polarimetric data obtained from them have been
utilized in several ways as complementary information to
the observations from optical and infrared region. Some
of the significant results include the detailed investigation
of lunar swirls, developing theoretical models for radar
scattering from the lunar regolith and analysis of several
new impact melt flows, etc, that were not observed in prior
radar imaging (Neish et al., 2011; Fa et al., 2011; Raney
et al., 2012; Saran et al., 2012; Carter et al., 2012; Neish
et al., 2014).
Operating with only one transmitting antenna polariza-
tion, hybrid-polarimetric SAR only uses half of the space
of polarization information provided by fully polarimetric
SAR. This limits the efficiency of hybrid-polarimetric SAR
in the exploitation of both wave and target Poincare ́ sphere
parameters. These parameters generated from fully polari-
metric SARs permit a complete characterization of target
scattering type and heterogeneity for the optimum extrac-
tion of target geophysical parameters.
To improve our ability to detect concentrations of ice,
both the radar backscattering coefficients and CPR should
D. Putrevu et al. / Advances in Space Research 57 (2016) 627–646 631
be analyzed simultaneously (as opposed to solely the CPR,
as demonstrated in the work of Campbell et al. (2006) and
Spudis et al., (2010a), (2013), etc). Fully polarimetric radar
scattering theory might be another alternative to detect ice
since it contains all the scattering information for any
arbitrary polarization state (e.g., circular, linear and hybrid
modes), more than a single parameter of the CPR (Fa
et al., 2011), and will be crucial in constraining the ambigu-
ities in detecting ice.
In addition to the prime application of polar-ice detec-
tion, a host of other applications can be addressed using
fully-polarimetric data, as discussed here. Fa et al. (2011)
developed a theoretical model for radar scattering from
the lunar regolith layer using Vector Radiative Transfer
(VRT) theory of random media (Jin, 1994). This model is
a fully polarimetric scattering model for both monostatic
and bistatic radar observations, which allows us to calcu-
late both the backscattering and bistatic radar scattering
coefficients for any desired transmit and receive polariza-
tion combinations. With the radar scattering model
developed in this study, a significant range of lunar surface
and subsurface properties can be estimated, such as lunar
surface roughness, subsurface rock abundance, regolith
thickness, and regolith dielectric properties. For example,
the regolith thickness is known at the Apollo landing sites
(Fa et al., 2011), which gives us a possibility of calibration,
leading to a much more accurate global regolith thickness
map with higher spatial resolution from Chandrayaan-2
fully polarimetric SAR data.
For smooth lunar regions with few buried rocks, such as
pyroclastic deposits, the ratio of the radar echo strengths in
orthogonal polarizations is sensitive to the real part of the
dielectric constant. Using the full-polarimetric SAR data
in combination with the above scattering model, the real part
of the dielectric constant could potentially be estimated over
large regions from orbit. The loss tangent of the same regions
could then be obtained from radar observations at L and S
band of Chandrayaan-2 SAR. With the derived dielectric
Some results from RISAT-1 Hybrid Polarimetric Mode. In these images, blue, red and green represent odd-bounce, even-bounce and volume-
scattering, respectively. (a) Intermixing of freshwater from Sabarmati river (Gujarat, India) with saline water of Arabian Sea. (b) Backscattering from sea-
surface due to rain over Andaman sea (cloud-like appearance). (c) Fresh lava flow from volcano Mt.Sinabung, Indonesia, as imaged on 8th July, 2015 (a
lower look angle of 34.5 resulted in foreshortening of the volcano). (d) Ahmedabad region, India, with incidence angle of 37. (e) Ahmedabad region,
India, with incidence angle of 21. Odd-bounce effects (in blue) are accentuated in image with lower incidence angle, compared to that in (d). (For
interpretation of the references to color in this figure caption, the reader is referred to the web version of this article.)
632 D. Putrevu et al. / Advances in Space Research 57 (2016) 627–646
properties, it might be possible to study how ilmenite content
(FeO and TiO2 abundances) varies with depth, which is not
easily investigated using other remote sensing techniques.
In summary, fully polarimetric SAR data at two fre-
quencies enables quantitative investigation of a variety of
surface and subsurface properties.
3.3. Resolution options
The slant-range resolution of the instrument is selectable
from a wide range of choices spanning 2–75 m, to address a
number of scientific objectives. A coarse resolution acquisi-
tion with high Signal-to-Noise Ratio (SNR), equivalent to
Noise Equivalent Sigma Zero (NESZ) of about 30 dB, is
necessary for certain applications like radio-physical char-
acterization of the regolith, and detection and estimation of
water–ice deposits. Finer resolution data (2 m or 3 m slant-
range, though with poorer NESZ) will permit derivation of
structural information from the lunar surface, particularly
from the regions inside the craters, for geo-morphological
studies, as discussed in the previous section. Options of
hybrid-polarimetry and full-polarimetry exist for all the
resolution modes of the L&S-band SAR, and the
polarization-resolution combination will be user selectable
through telecommand.
3.4. Simultaneous and standalone SAR modes
One of the salient features of the dual-frequency SAR is
simultaneous L- & S-band operation, in addition to the
stand-alone operation in the individual bands. Simultane-
ous data gathering in the two bands of operation allows sur-
face roughness and dielectric constant estimation without
recourse to any other observations. The sensitivities of fre-
quency of illumination and the look angle to the surface
roughness are different for L-band and S-band observa-
tions, a property variance that can be effectively exploited
in this application. As discussed in the previous section on
science goals, multi-frequency data are of significant value
for estimating lunar polar-ice detection index, required for
detection and estimation of water–ice deposits. The index
based on a multi-layer scattering model for radar backscat-
ter at L- and S-bands showed that buried ice and rock inclu-
sions are separable in terms of their radar backscatter at
different incident angles (Pandey et al., 2013b). This index,
along with the other important parameter CPR, will be very
useful for identifying and characterizing lunar polar water–
ice. Simultaneous imaging in L- and S-bands is further
expected to improve the estimation accuracy of this index.
An important feature of simultaneous mode of acquisi-
tion is the targeted phase coherency, better than 45
between L- and S-band data corresponding to each target,
using synchronization signals between the systems for the
two frequency chains. In addition to these, use of onboard
chirp replica for range compression is mandatory to
achieve the required phase coherency. Synchronization of
this order may not be possible with time-separated L- &
S-band data-takes over the same region of interest, thereby,
calling for simultaneous mode of imaging.
3.5. Axial ratio
An axial ratio of better than 2 dB has been aimed at for
this system, which will be obtained by ensuring minimum
amplitude and phase imbalances between H and V trans-
mission paths (to be eleborated later) and by having a good
cross-polarization ratio of the antenna (better than 30 dB).
This axial ratio is found to be adequate for the targetted
mission science. For example, CPR, the most useful param-
eter used to detect the presence of water–ice at the lunar
poles, is found to be far less affected by the transmitted
axial ratio than by the polarimetric properties of the lunar
surface (Raney et al., 2011). Although other Stokes-based
measurements may be more sensitive to imperfect circular-
ity of the illuminating field, recent studies indicate that for
a dominant scattering mechanism, the associated decompo-
sition parameter is less sensitive to variations of transmit
ellipticity (Sabry and Vachon, 2014).
As the study of effect of imperfect-circularly polarized
transmission on retrieved polarimetric parameters is a
new topic, the validation of results obtained is not accom-
plished yet. Existing results related to such kind of analyses
are not based on original hybrid-polarimetric data (Touzi
and Charbonneau, 2014). Work is in progress on simula-
tions using varying degrees of ellipticity in the transmitted
field, against a variety of known target types, including, in
particular, ones that have a dominant linearly-polarized
component at an angle with respect to H or V
polarizations.
3.6. Radiometer mode
This mode of operation will be used to measure the
brightness temperatures of the surface from which regolith
thickness and distribution may be estimated, as discussed
in the previous section. The empirical relations to derive
the same are, at present, under development. This mode
has been added at a late stage of development of the system
using only software provisions, without any implications
on the hardware design and configuration; further details
on this are presented in Section 4.4.
The incorporation of the above features in the system
concurrent with the stringent physical constraints from
spacecraft design posed many challenges that were tackled
with ingenuity. In effect, two complete SAR systems were
realized in a 15 kg total mass budget. Constraints on
data-rate (of 160 Mbps) and raw-bus power (of 100 W),
were met irrespective of any resolution and polarization
settings and in either standalone or concurrent (simultane-
ous L&S-band operation) modes of operation. The system
design efficiently marries the science requirements and the
demanding spacecraft constraints, as described in the fol-
lowing section. At the end of the section, Table 3 compares
the salient features of Chandrayaan-2 dual-frequency SAR
D. Putrevu et al. / Advances in Space Research 57 (2016) 627–646 633
with those of recent instruments to the Moon, MiniSAR
(Chandrayaan-1) and MiniRF (LRO).

- System configuration
The dual-frequency SAR will operate from a 100 km
circular polar-orbit of the Moon, in the dawn-dusk season,
when other optical instruments (sharing the Chandrayaan-2
resources) do not operate. Incidence angle coverage from
9.6 to 36.9, on either-side of the sub-satellite track,
will be achieved by roll-tilting the spacecraft. The SAR
will operate in conventional stripmap mode with a 10 km
swath, and a selectable slant-range
resolution (from 2 to 75 m, in eight discrete steps). The
data acquisition period is about 8 min in each 2-h orbit,
during the dawn-dusk seasons.
The instrument is configured as two independent and
physically separate systems, L-band SAR and S-band
SAR, sharing a common antenna. This antenna also serves
S-band telemetry and telecommand (TTC) requirements of
the lander. The selection of functionality is effected by
means of two switches provided in V & H-polarization
paths. This is possible since the opera-
tion of the SAR and the communications subsystem are
mutually exclusive. A 0/90 hybrid is used to convert sig-
nal from linear to circular polarization, required for lander
TTC. It is important to note that this hybrid is only in the
path connecting SAR antenna and lander TTC system. Cir-
cular polarization transmission of SAR (required for
hybrid polarimetry) is effected by digital phase-shifters in
the SAR transmitters, by providing relative phase offset
of 90 between H and V chains. This aspect is further elab-
orated under Section 4.2 describing transmit chain of the
system. The L-band counterpart has an identical configura-
tion but for the switches and hybrid for Lander TTC.
4.1. Antenna
The antenna is a planar-microstrip array of dimensions
1.35 1.1 m, designed for dual-band and dual-polarized
configuration. The L-band and S-band patches and distri-
bution networks are interleaved on different layers to meet
the gain and bandwidth requirements. Both L- and S-
bands of the SAR need a bandwidth of 75 MHz; in the case
of the S-band, however, the lander TTC frequencies neces-
sitate an overall bandwidth of 180 MHz for the antenna, as
illustrated . The antenna is highly optimized for
mass and weighs a mere 3.6 kg, while meeting the above-
mentioned performance requirements.
4.2. Transmit chain
The linear frequency modulated (‘chirp’) signal for
transmission is generated by Digital Chirp Generator
(DCG) section at baseband frequencies, in complex (in-
phase and quadrature-phase or ‘I and Q’) form, corre-
sponding to the selected resolution option. The I and Q
components are vector modulated in the Frequency Gener-
ator (FG) unit to the transmit band. The signal is divided
into two paths for V and H chains, for further amplifica-
tion and transmission. The Solid State Power Amplifier
(SSPA) chains are provided with 6-bit digital phase-
shifters to correct any phase-imbalances between the two-
chains. An additional phase-shift of 90 in one of the
chains is provided to achieve circular transmission, during
hybrid polarimetric mode. These phase-shifts are applied
after compensating for temperature-induced variations to
maintain the axial ratio of circularly polarized signals.
The phase compensations are applied based on ground-
characterized data held in look-up tables (LUTs) in the
system. Thermistors located on the SSPAs provide temper-
ature for proper selection of phase from the LUT.
The transmitter package comprises SSPA(V) and SSPA
(H) chains, along with their dedicated Electronic Power
Conditioners (EPC). The SSPAs are realized using Gallium
Nitride (GaN) devices in Class-F design, in order to
achieve a high efficiency of the order of 45%. This is critical
in view of the raw power limitations.
A fraction of the SSPA output signal is coupled directly
to the receiver for chirp-replica acquisition to enable range-
compression. A 1:2 combiner/divider network, part of the
transmitter package, combines H&V coupled signals first
and then divides equally before feeding to the coupled
ports of the receivers; this is necessary for acquiring the
replica in all the four polarization combinations of HH,
HV, VV and VH, and is critical for polarimetric
applications. It is also important to note that these combi-
nations necessitate critical excitation and acquisition tim-
ings to avoid intermixing of H & V components. Every
imaging session is preceded and followed by a replica
acquisition. A separate mode dedicated for calibration is
also planned.
During the initial phase of design, 2.25 GHz was
selected as S-band SAR center-frequency, with 75 MHz
bandwidth. As this frequency band was found to be inter-
fering with TTC downlink frequencies (indicated in Fig. 9),
it was shifted to a center-frequency of 2.5 GHz, after sim-
ulation results indicated no particular disadvantages in per-
formance. This was later validated during in-lab
characterization of prototype hardware. Both L-band and
S-band frequencies are multiples of 250 MHz, as they are
being derived from a basic 250 MHz TCXO (Temperature
Compensated Crystal Oscillator) source; this also explains
the quantum of shift in frequency selection of S-band SAR
from 2.25 to 2.5 GHz.
4.3. Receive chain
The low noise and high gain receivers are equipped with
filters (some of them sharp due to the presence of close-by
frequencies for S-band TTC link to Earth shown in Fig. 9)
at the input to provide sufficient rejection to the coupled
out-of-band signals from different sources. The filtered sig-
nal is then amplified and I-Q demodulated. A 6-bit digital
attenuator with a dynamic range of about 30 dB is also
provided to keep the signal input to the Analog-to-
Digital Convertor (ADC) within optimal range. 8-bit digi-
tization is implemented with selectable sampling of either
83.3 MHz (for 75 MHz bandwidth corresponding to 2 m
resolution) or 62.5 MHz (for all other bandwidths).
A hardware anti-aliasing filter has been used in the recei-
ver corresponding to the highest bandwidth signal. The
additional filtering necessary for lower bandwidth modes
has been implemented digitally (prior to decimation).
Onboard processing includes matched-filter based range-
compression by using the chirp replica from pre-imaging
acquisition; this reduces the data volume. However, to
meet the final data rate limit of 160 Mbps, further compres-
sion of the data is necessary. The signal, after onboard
range compression (but prior to azimuth compression
planned using the ground processor) preserves gaussian
characteristics and therefore, allows the application of a
Block Adaptive Quantization (BAQ) process to reduce
Fig. 9. S-band frequencies existing on Chandrayaan-2 orbiter. SAR and
Lander TTC use a common antenna.
D. Putrevu et al. / Advances in Space Research 57 (2016) 627–646 635
the downlink data volume. The BAQ setting is selectable
from 2- to 6-bits output.
Meeting the physical goals for the accommodation of
the receivers meant a great deal of miniaturization in the
two receivers (for V & H) and frequency generator, realized
in a single tray. Indigenously developed Microwave Inte-
grated Circuits (MICs), Monolithic Microwave Integrated
Circuits (MMICs) and Multichip Modules (MCMs) were
used extensively to achieve the mass and volume targets.
The digitally implemented functions in the payload are
mainly three, viz. Payload Controller (PLC), Digital Chirp
Generator (DCG) and Data Acquisition & Compression
System (DACS). Traditionally, these functionalities have
been in separate subsystems or packages; however in this
payload they have been integrated into a single-board
high-density entity using Field Programmable Gate Array
(FPGA, Xilinx Virtex 4) devices and Application Specific
Integrated Circuits (ASIC) thereby achieving significant
gains in mass and volume. The chirp-generation is pro-
grammable based on ground-command, depending on the
selected options of bandwidth (2–75 MHz) and pulsewidth
(25–80 ls).
The payload controller runs on a microcontroller device,
onboard controller (OBC) ASIC, in the single-board digital
subsystem. The PLC takes care of all the control and tim-
ing signals necessary for the subsystems to tick in unison.
The PLCs of the L-band and S-band instruments intercom-
municate and manage the synchronization required during
L&S-band simultaneous mode of operation. The integrated
Receiver-FG and Digital subsystems have a common EPC,
stacked with these two packages.
4.4. Radiometer mode of operation
During the radiometer mode of operation, the RF trans-
mission is inhibited and the position of the receive windows
is set corresponding to the observation geometry. The
introduction of this mode has been possible at an advanced
stage of system realization owing to the fact that the pair of
receiver protection switches are absorptive in nature, and
therefore, will be useful for ‘‘hot-load calibration” required
for reliable radiometer measurements. Out of the two
protection switches at the input of a single receiver chain
(H/V), only the one which is external to the receiver is shown
in the block schematic. The pair of switches is oper-
ated by a common command from PLC. During the pro-
tection (isolation) state of the switches, the antenna is
isolated and each switch is connected to the RF matched
termination and can be used for ‘Noise-only’ measurement
(N) like in a Dicke-configuration. For ‘Signal + Noise’
measurement (S + N), the switch-pair is in insertion-state
(connects the antenna), and enables acquisition of
antenna-temperature measurements. Antenna temperature
is the gain-weighted sum of brightness temperatures as seen
from the antenna. The ‘S + N’ and ‘N’ measurements are
averaged for 100 ls onboard and downlinked for further
averaging on ground to improve the temperature sensitivity.
An important aspect of the radiometer mode is the need for
correction for emissions from front-end components
between the antenna and the receiver. This calls for temper-
ature measurements at all such elements, viz, switches at
receiver input, circulators (and the hybrid in case of S-
band), which form part of the SAR auxilliary data stream.
Prior to the start of the radiometer mode, the spacecraft
will be yaw-rotated by 110 for cold-sky measurements to
improve the accuracy of brightness temperature estimates.
The frequency of such measurements is yet to be finalized.
The achievable antenna temperatures and brightness tem-
perature accuracies are also under detailed study, but are
expected to be of the order of 2 K.
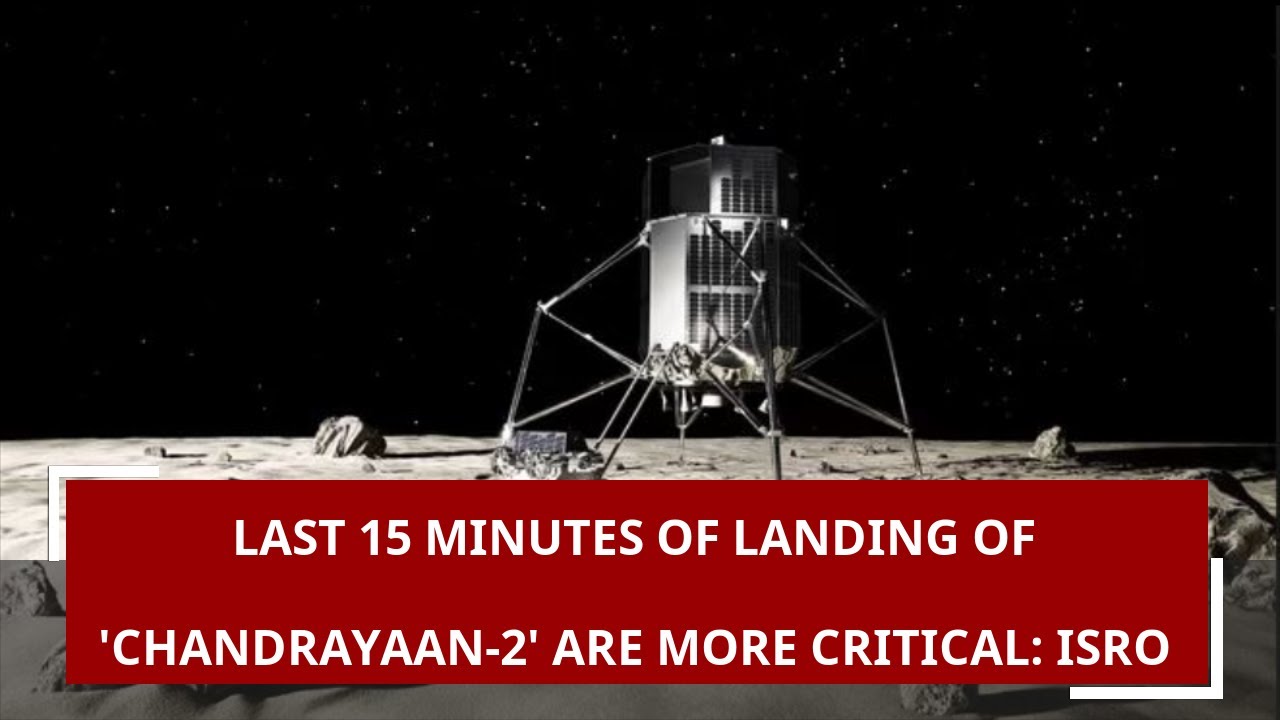
- System design
As a departure from the general flow of SAR system
design, in which antenna area requirements are derived
from system specifications, the Chandrayaan-2 SAR design
had to start with antenna area as an input. This was due to
severe payload accommodation and mass constraints. The
maximum possible aperture size for the spacecraft was
1.35 1.1 m. The system design envisaged multiple resolu-
tion modes from 2 m to 75 m forcing a wide range of signal
bandwidths from 75 MHz to 2 MHz with attendant chal-
lenges in the hardware. In addition, the available power
and data rate were limited. Based on these and the target-
ted NESZ performance, several trade-off studies were per-
formed among factors like transmit power, pulsewidth,
PRF and other operational parameters for stand-alone
and simultaneous operation of the two bands. Variable
pulsewidth (from 25 ls to 80 ls, in steps of 5 ls) became
a necessity to balance instrument performance with avail-
able spacecraft resources. The SAR performance was opti-
mized over a swath of 10 km, in both hybrid as well as full
polarimetric operation. In full-polarimetric mode, how-
ever, the maximum pulsewidth possible was found to be
50 ls, as the echo data-window had to be accommodated
in shorter inter-pulse periods (owing to higher receive
PRF). This resulted in a penalty on radiometry (NESZ
degradation 2 dB).

Onboard signal processing, using range-compression
and BAQ, was planned to meet the allowable data-rates.
The range delay variation over the 10 km swath is 11 ls
to 41 ls, leading to echo-window (including transmitted
pulsewidth) variation of 36 ls to 121 ls. As the pulsewidth
forms a significant fraction of the overall echo-window,
some reduction in data rate (38–70%) was possible by
onboard range-compression. The BAQ achieves further
compression of the data and at the nominal setting of 4 bits
yields a maximum final compressed data rate of 160 Mbps
while meeting all the mission goals.
As has been mentioned earlier, configuring a dual-
frequency SAR called for introduction of filters at receiver
front-ends to reject out-of-band signals, thereby increasing
receive-chain losses and impacting system SNR adversely.
This is more critical for the S-band SAR, as the S-band
TTC frequencies (communication link to the Earth) are
very close to the SAR frequencies and are constantly pre-
sent. RF coupling analysis was carried out to determine fil-
ter rejections required for acceptable leakage during SAR
operation and for safety of SAR receivers during periods
of non-operation. Similarly, spurious levels from SAR
transmit chain have been specified to constrain coupling
to TTC receiver to acceptable levels.
The system specifications resulting from the foregoing
considerations and trade-offs are presented in Table 1.
range of target back-scatter properties resulting in SNRs
of 0–10 dB, respectively. Radiometric accuracy is specified
to be better than 1 dB.
Since the radar echo windows need to avoid transmit
pulse durations (and nadir returns), and range and azimuth
ambiguities need to be minimized, analyses were carried
out for feasible PRF ranges. For this, a performance metric